I am interested in planet formation theory, particularly how feedback between protoplanetary disks and embedded planets affect the planet formation process. My research focuses primarily on radiative transfer in protoplanetary disks. I run computationally intense numerical models that show how planet-disk interactions can change a growing planet's formation environment. I also use my theoretical models to predict observable signatures of planet formation in disks.
- Radiative Transfer in Planet-Forming Disks
- Protoplanet Shadows
- Core Accretion vs. Disk Instability
- Axisymmetric Gaps
- Planet Formation in Close Binaries
- Planet Migration
- Superheated Inner Rims and Transitional Disks
- Astrobiology
Radiative Transfer in Planet-Forming Disks
In the later stages of star formation, the envelope surrounding the nascent star dissipates, and the remnant circumstellar material forms a disk that passively accretes at a very slow rate (≤10-7 Msun yr-1) onto the star. This is the era of planet formation. These disks are gas-dominated and optically thick, and are often referred to as primordial or protoplanetary disks to distinguish them from the optically thin dust-dominated debris disks seen around older stars. Stellar irradiation is a significant source of heating in these disks, causing a temperature inversion at the disk's surface and setting the temperature of the disk's photosphere.
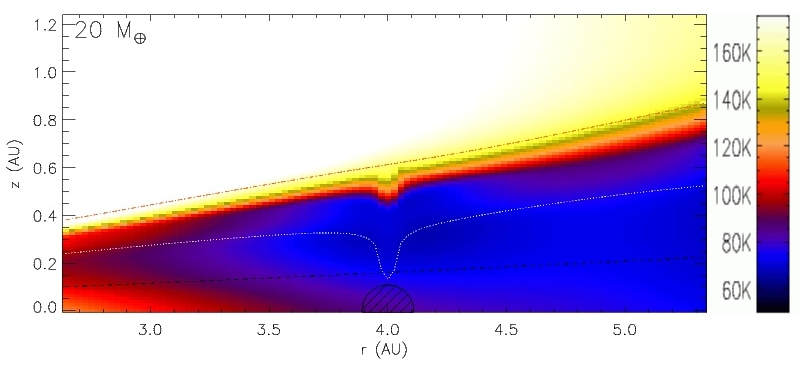
Slight perturbations to protoplanetary disks, such as those induced by embedded planets, can significantly change the temperature structure of the disk photosphere as patterns of shadowing and illumination at the disk surface shift (Jang-Condell 2004; Jang-Condell & Sasselov 2003, 2004). Additionally, self-consistently modeling the temperature and density variations of the disk is important because shadowed regions cool and contract while brightened regions heat and expand, creating a positive feedback loop (Jang-Condell 2008). I developed a semi-analytic method of calculating radiative transfer in an optically thick disk that takes advantage of an analytical solution to radiative transfer in a plane-parallel semi-infinite slab (see Calvet et al. 1991; D'Alessio et al. 1998, 1999; D'Alessio, Calvet, & Hartmann 2001, and references therein).
Protoplanet Shadows
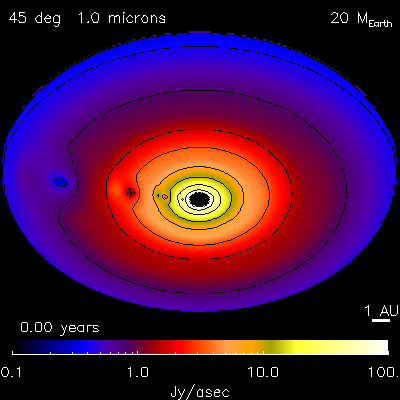
(Click here for preprint)
Cores of giant planets are expected to grow to about 10-20 Mearth before they begin to accrete gaseous envelopes. These objects are generally not massive enough to clear a gap in a disk and should therefore only produce local perturbations to the density and temperature structure of the disk. Their gravitational potentials locally compress the disk material, effectively creating a dimple at the surface. The side of the dimple closer to the star will be shadowed while the far side is brightened, much like the bowl of a crater. This results in a shadow at the position of the planet, with a bright spot on the far side of the planet. The size of the perturbation varies with the mass and position of the planet (Jang-Condell & Sasselov 2003, 2004; Jang-Condell 2008). The feature is prominent in scattered light and in mid-infrared wavelengths, corresponding to thermal emission from the surface layers of the disk. However, since the shadowing effects are most prominent in the upper layers of the disk, the feature becomes washed out in radio wavelengths, which probe closer to the disk midplane (Jang-Condell, in prep).
Recent scattered polarized light observations
of the Herbig Ae star AB Aur show evidence of a shadow in the disk.
that could indicate the presence of a planet
(Oppenheimer, et al. 2008).
The size and contrast of the shadow are consistent with
a 1 MJup planet at 100 AU from star
(Jang-Condell & Kuchner, in prep).
Core Accretion vs. Disk Instability
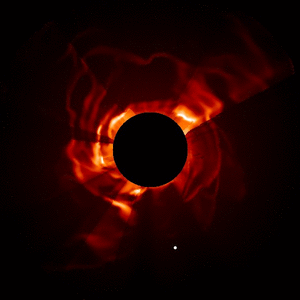
One major debate in planet formation theory is whether giant planets form through disk instability or core accretion. In the disk instability scenario the protoplanetary disk fragments into planet-mass clumps, whereas in core accretion, smaller bodies coagulate into larger and larger bodies until a core massive enough to accrete a gaseous envelope forms. Signatures of core accretion are described above, but what would disk instability look like? Using snapshots from a 3D hydrodynamic simulation (Boss 2001), I calculated simulated images of a disk undergoing disk instability (Jang-Condell & Boss 2007). Since scattered light traces out diffuse density perturbations at the surface of the disk rather than the overall structure of the disk, the position of the forming planet cannot be seen at optical wavelengths. Radio wavelengths, where the disk becomes optically thin, are better for probing the position of the planet-forming clump (Jang-Condell & Boss, in prep). Another signature of disk instability is that the scattered light images change on times scales of a few years because of stirring by the forming planet.
>
Axisymmetric Gaps
Resolved HST images of the T Tauri star TW Hya in scattered light show evidence of a developing gap in the disk (Debes, Jang-Condell, et al., in prep). The surface brightness profile can be successfully modeled simultaneously across multiple wavelengths as as a partially cleared gap, possibly caused by an embedded planet. As a planet grows in mass and approaches the gap-opening threshold, it progressively clears more and more material from an annulus along its orbital path. These partial gaps, as well as full gaps from massive planets, are subject to shadowing and illumination effects just like planet-induced dimples, so the radiative feedback must also be appropriately treated.
Planet Formation in Close Binaries
Close binary stars are an interesting laboratory for studying planet formation because binary companions should truncate a planet-forming disk (Artymowicz & Lubow 1994). By modeling a truncated protoplanetary disk in a close binary system, we can learn how planets in such extreme environments form. My research shows that planet formation in the hierarchical triple HD 188753 cannot occur in situ (Jang-Condell 2007). Indeed, the claim of of a planet in this system (Konacki 2005) has since been refuted (Eggenberger, et al. 2007). On the other hand, I find that formation of the planet in γ-Cep (Hatzes, et al. 2003) is perfectly reasonable (Jang-Condell, Mugrauer, & Schmidt 2008). I also argue that planet formation in close binaries is more likely to occur through core accretion than disk instability because it is difficult to get truncated disks that are simultaneously both massive and cool enough to be gravitationally unstable.
Planet Migration
Interactions between forming planets and protoplanetary disks should lead to inward migration. In Type I migration, planets below the gap-forming threshold move rapidly inward through the disk, whereas in Type II migration, massive gap-opening planets become locked to the disk and move inward on the visocus accretion timescale. My own research into Type I migration shows that migration rates are sensitive to the temperature gradient in the vicinity of a planet, and temperature perturbations induced by planet shadows can slow the migration rate (Jang-Condell & Sasselov 2005).
On the other hand, if AB Aur and TW Hya do in fact harbor planets as suggested above, how would these planets get to distances of 80-100 AU if migration moves planets inward? This is an question of both theoretical and observational interest. Under what conditions to planets migrate inward versus outward? Other types of migration have been proposed, such as corotation torques in asymmetric gaps and turbulent migration. In order to test these hypotheses, we must discover the frequency of distant planets. Definitive signatures of planets in disks, such as the ones I work to model, will help constrain these statistics. If planets are likely to exist at ~100 AU from their parent stars, this increases the possibility of directly imaging young hot planets.
Superheated Inner Rims and Transitional Disks
Many disks are observed to have inner holes, some corresponding to the dust sublimation radius (e.g., Eisner et al. 2005; Eisner, Chiang, & Hillenbrand 2006), and others several AU in size (Forrest et al. 2004; D'Alessio et al. 2005; Uchida et al. 2004; Bouwman et al. 2003; Rice et al. 2003). The latter group is sometimes referred to as transitional disks, meaning that they exhibit characteristics of both optically thick and optically thin disks. The inner truncated edge of these disks are fully illuminated by stellar irradiation rather than at grazing incidence. This causes the inner walls to be heated above the temperature of a continuous disk at the same radius, and therefore be puffed up to greater heights. Does the outer disk behind the puffed up inner wall become self-shadowed and collapse (Dullemond, Dominik, & Natta 2001; Dullemond & Dominik 2004a,2004b), or is there enough light scattered from the upper layers of the disk to heat the interior (D'Alessio, et al. 2006)? The models cited above are 1+1D models, meaning that they treat vertical (perpendicular to the midplane) radiative transfer, but not in the horizontal or radial direction. My radiative transfer methods do not differentiate between radial and vertical directions, but rather depend on the the three-dimensional geometry of the disk. I can accurately model radiative transfer regardless of orientation and I expect to settle the debate over self-shadowing in disks with inner clearings.
Astrobiology
I am interested in studying the properties of exoplanet systems that might allow habitable worlds to exist. I have researched the effect on the formation and sublimation of water ice in the vicinity of protoplanet shadows (Jang-Condell & Sasselov 2004; Jang-Condell, Podolak & Sasselov, in prep). Water ice has important consequences on the growth and evolution of planets embedded in disks, in addition being an essential ingredient for life. I plan to further study the dynamics of water delivery to emerging protoplanets through use of detailed hydrodynamics simulations.